HEDS Research Area
Material Properties at Extreme Conditions
- Home
- Research
- Research Areas
- Material Properties at Extreme Conditions
Study of the properties of materials under extreme conditions supports research in multiple fields, including planetary geophysics, materials science, and inertial confinement fusion. Complex physics interactions across a variety of length and time scales must be understood in regions that are difficult to access and diagnose—with time scales ranging from picoseconds to billions of years, and length scales ranging from atomic to bulk. This research involves studying several properties of HED matter, including equation of state, opacity, and charged-particle transport.
Dive deeper into our research
Creating and Studying HED Matter
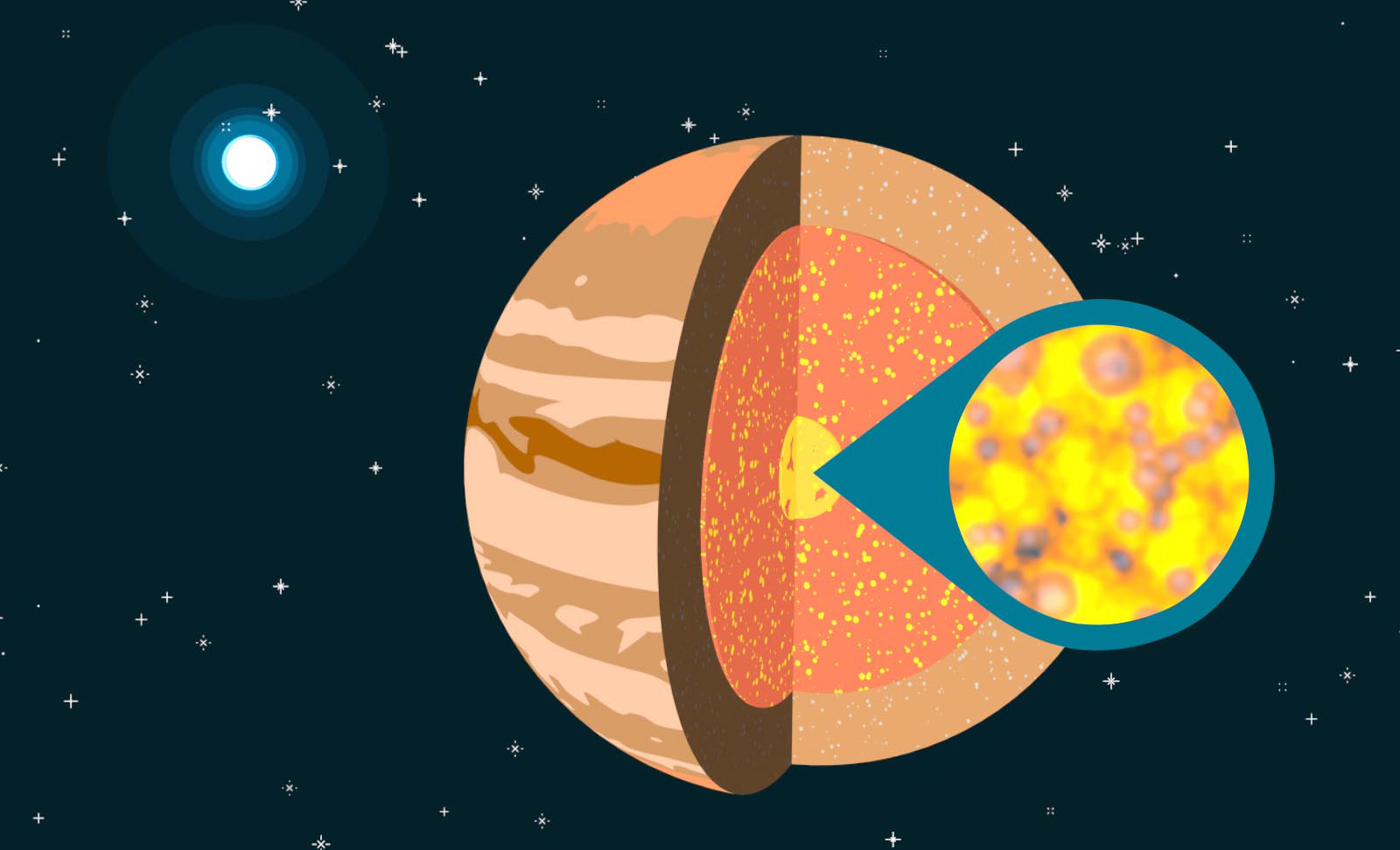
At LLNL, we create and study two forms of HED matter—warm dense matter (WDM) and hot dense matter (HDM). Creating these states is extremely challenging. Large amounts of energy must be delivered in short periods of time via lasers, electron or ion beams, or shocks. These sudden changes produce dynamical plasmas that are often out of equilibrium. The electrons and ions may be described by different temperatures, or the electrons themselves may not thermally populate the atomic levels.
The National Ignition Facility (NIF) at LLNL is one of the few facilities on Earth capable of producing HDM. In many NIF experiments, deuterium and tritium is crushed to a thousand times solid density and to tens of millions of degrees Kelvin—conditions similar to the center of the Sun. In this regime, nuclear fusion adds energy to the system, many hydrodynamic instabilities grow, and thermal radiation dominates over charged-particle energy transport.
When we study HDM, we try to understand how to more effectively produce this state, how the fusion heating affects its dynamics, how compressible the material is, and the behavior of atoms embedded in it. LLNL scientists have designed sophisticated diagnostics to measure neutrons, x-rays, and charged particles emanating from the HDM before it rapidly explodes. They employ detailed multiphysics radiation hydrodynamic simulations to predict and understand HDM dynamics.
WDM is near-solid-density material at temperatures ranging from a few to hundreds of electron Volts, and at pressures near a megabar. It is found at the center of giant planets and brown dwarfs—and it is produced during HED experiments at LLNL. It is not defined by any discontinuity in thermodynamic quantities, so is not formally a state of matter. Rather, it is best defined as a research frontier for material properties.
Different specialists approach the study of WDM with different types of questions. For example:
- A chemist might ask, “How do the high temperatures affect the electronic state and its free energy?”
- A plasma physicist might want to understand: “How do strong Coulomb interactions, partial degeneracy, and large fluctuations affect the response of the material?”
- A materials scientist might ask, “What exotic or new phases may I see at high pressures?”
- An atomic physicist might explore: “How do the electric microfields and their fluctuations affect atomic transition lines and edges?”
These questions hint at the diversity of lines of inquiry about WDM. The field is at the intersection of quantum mechanics, plasma theory, many-body theory, and thermodynamics.
Learn More:
- Study sheds light on elusive warm dense matter
- First experimental evidence for superionic ice
- New research re-creates planet formation, super-Earths, and giant planets in the Laboratory
Contact
- Paul Grabowski
- grabowski5 [at] llnl.gov (grabowski5[at]llnl[dot]gov )
Equation of State
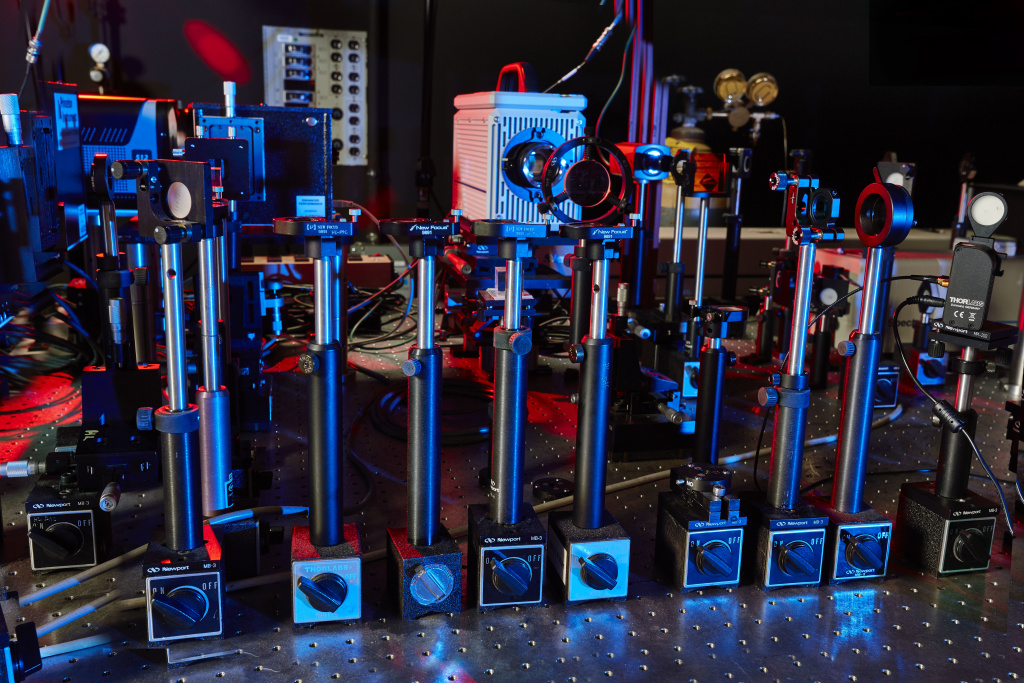
From the nearly instantaneous detonation of a nuclear weapon or high explosive, to the evolution of a planet’s core over billions of years, a material’s equation of state (EOS)—which provides the relationship between its pressure, temperature, and density—is required to understand its dynamics. LLNL researchers determine accurate EOS data of key elements to inform computational models of material behavior. These models drive simulations that demonstrate how materials respond to enormous pressures and high temperatures.
Scientists conduct experiments at LLNL’s National Ignition Facility (NIF) to determine EOS data for solid materials at pressures up to 5 terapascals (TPa), or 50 million times Earth’s ambient air pressure. For decades, the traditional method for determining a material’s EOS was to send a sudden shock through a material and measure its response. This shock compression method relies on lasers, gas guns, and other tools to launch a virtually instantaneous shock wave through a sample, rapidly melting it. However, shock compression limits the combination of pressures, temperatures, and densities that may be reached to a narrow portion of the material’s EOS. To reach a broader set of conditions, an alternative route—ramp compression—is proving immensely useful.
Ramp compression experiments on NIF apply a carefully tailored laser pulse shape that more “softly” compresses a material without forming a shock. The technique helps scientists better understand the physics of solids compressed to extreme densities under a wider range of pressures and much lower temperatures.
Another method used to determine EOS without imparting a violent shock involves small diamond anvil cells (DACs), a technique LLNL researchers helped to pioneer. DACs compress micrometer-sized samples between two brilliant-cut diamonds, generating pressures of several tenths of a TPa. DAC experiments last much longer than NIF shots—from milliseconds to minutes to even days—generating much lower temperatures. These lower temperatures generated by EOS ramp compression experiments make these tests highly relevant to the study of high-pressure physics considered to underlie the structures of stars and planets both in our solar system and beyond.
Learn More
- Gently compressing materials to record levels
- Scientists measure temperature under shock conditions
- New target facility will help unlock plutonium’s secrets
- The pressure’s on: Diamond anvil cells reimagined
- Lab team’s new interferometric instrument improves refractive index measurements at high pressure
- Record EOS measurement pressures shed light on stellar evolution
Contact
- Jon Eggert
- eggert1 [at] llnl.gov (eggert1[at]llnl[dot]gov)
- Phil Sterne
- sterne1 [at] llnl.gov (sterne1[at]llnl[dot]gov)
- Christine Wu
- wu5 [at] llnl.gov (wu5[at]llnl[dot]gov)
Opacity
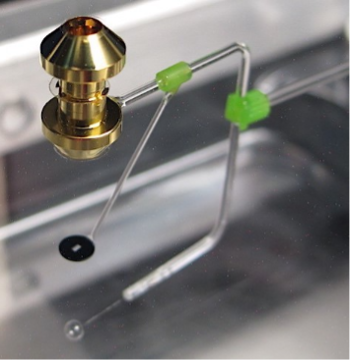
Stars, including our Sun, consist of hot, dense plasma at temperatures measured in millions of degrees. Fusion energy produced in the center of a star must flow out to the surface, first radiatively (as x rays) and then convectively (flowing plasma). Opacity, or the property of the plasma that limits the radiative x-ray flows, is determined by the ability of individual ions in the plasma to absorb and then re-emit those x rays. Opacity depends on the elements present (including impurities such as oxygen and iron), and the temperature and density of the plasma.
Opacity plays a role in two major ongoing scientific disagreements. The location of the boundary between the radiative and convective zones in the Sun has been measured and is not matched by the best available theoretical models. Separately, for fading white dwarf stars, there is doubt about the cooling rate—the rate of energy flowing out. The cooling rate of the oldest dwarf stars helps to set a lower bound on the age of the universe, but it depends on the opacity.
HED experiments produce plasmas at these stellar conditions, and we can directly measure the opacity by studying the transmission of x rays over a suitable range of wavelengths, roughly 0.1 to 0.6 nanometers. Supercomputers can calculate the opacity in great detail from the fundamental quantum physics of atom–photon interactions, as affected in myriad complex ways by the surrounding plasma.
Scientists at LLNL work with collaborators to measure the opacity of oxygen, iron and other materials at temperatures ranging from 1‒10 million Kelvin and densities from solid density down to 1% of solid density. Some of these experiments are conducted using long-pulse lasers (0.1‒10 nanoseconds), such as the National Ignition Facility at LLNL. In these experiments, one group of lasers is used to heat a special oven (a hohlraum) with a sample inside of it. The hohlraum has holes so that x rays from a broad-spectrum x-ray backlighter can pass through, or around, the sample. This produces a measurement of the opacity from the x-ray transmission—the ratio of the number of x-rays passing through the sample to those passing around it.
However, the long-pulse laser technique becomes problematic when the attenuating material is a plasma with bright emission in the spectral range of interest. Additionally, the plasma conditions need to be uniform over the duration of the measurement (i.e., minimal gradients in density and temperature). Short-pulse lasers provide a technique for inferring opacities using the plasma emissivity to infer opacity. Under the appropriate conditions (near thermal equilibrium), a physics principle called Kirchhoff’s law suggests the material emissivity is proportional to the inverse of the opacity. For short-pulse laser-heated solids, the rapid heating of the target results in a uniform, minimal gradient plasma at near-solid density for short durations (~20 picoseconds), making it optimal to apply Kirchhoff’s law. Both types of experiments play an important role in LLNL’s opacity program.
Learn More
Contact
- Robert Heeter
- heeter1 [at] llnl.gov (heeter1[at]llnl[dot]gov)
Charged-Particle Transport Properties
Charged-particle transport is a key part of HED plasma science. Transport coefficients feed into both radiation-hydrodynamic simulations and diagnostic data interpretation. They govern the transport of mass, momentum, and heat—where things are, how fast they move, how fast they slow down, and how their energy is exchanged and moved around.
There are two types of transport properties. The first is classical transport properties, such as ion diffusion and viscosity, for which the quantum electrons are bystanders screening the ions. The second is quantal transport properties, such as thermal and electrical conductivity, ion-electron equilibration, and stopping power, for which the response of the quantum electrons is directly related to the transport properties.
Classical transport properties are best calculated with molecular dynamics, in which each ion’s position and velocity is propagated according to Newton’s laws of motion. Of course, the forces between ions requires knowledge of how the electrons screen and bind to them. Once those are known, transport properties can be calculated indirectly by compiling statistics in density or current fluctuations or a non-equilibrium system can be directly evolved. Research on these processes focuses on accurate descriptions of inter-ionic forces and computational methods to scale to large system sizes.
Quantal transport properties require the calculation of the dynamic properties of the many-body plasma system. The underlying equation to solve is the time-dependent Schrödinger equation, which quickly becomes infeasible for large numbers of electrons. Thus, theoretical research focuses on developing and testing approximate models and equations. Experimental challenges include the creation and diagnosing of these hard-to-reach plasma systems.
Learn more
Contact
- Paul Grabowski
- grabowski5 [at] llnl.gov (grabowski5[at]llnl[dot]gov )